Starting Small: Can Hydrogen Propulsion Grow Out Of Its Initial Niche?
When Universal Hydrogen closed its doors in June after failing to secure more funding, it raised questions about the viability of introducing zero-emission hydrogen propulsion to commercial aviation by first retrofitting regional turboprops.
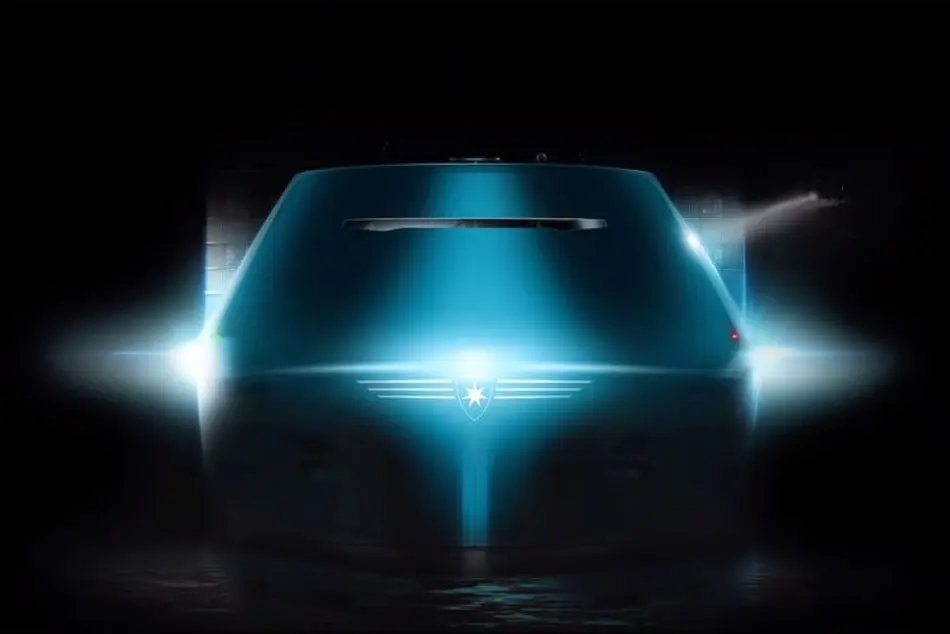
When Universal Hydrogen closed its doors in June after failing to secure more funding, it raised questions about the viability of introducing zero-emission hydrogen propulsion to commercial aviation by first retrofitting regional turboprops.
Others have rallied in defense of the strategy to start small and then grow upward and outward. But the question remains: If you begin in a narrow niche—technological, operational or geographical—how do you cross the divide into mainstream markets?
Universal Hydrogen chose a single target within the regional aircraft market—the ATR 72—with which to prime the pump for a business model based on supplying green hydrogen. The company elected to work with suppliers to develop its hydrogen-electric powertrain, acting as the integrator.
Others have taken a different approach. They range from Cranfield Aerospace Solutions (CAeS), which is beginning with a conversion of the nine-passenger Britten-Norman Islander to hydrogen fuel-cell propulsion, to Airbus, which is eyeing a clean-sheet hydrogen-electric 100-seater for 2035 service entry.
Then there is ZeroAvia, which is converting the nine-passenger Cessna Caravan but went a step further to bring the key fuel cell, electric motor and power electronics technology in-house so it can develop more powerful and efficient hydrogen-electric powertrains for regional jets and even single-aisles.
CAeS has completed the design of a 240-kW hydrogen fuel-cell powertrain that fits within the nacelles of the Islander, a popular aircraft for island-hopping and connecting remote and rural communities. But how does the UK company see the technology growing out of this modest niche into larger markets?
“By getting a small aircraft into a small operation, you’re driving more than the aircraft technology,” CAeS Chief Strategy Officer Jenny Kavanagh says. “You’re proving what the technology can do. You’re proving how you can certify it on an aircraft and make it safe, but at the same time, it’s not just the aircraft—it’s also the ecosystem. Airports and airlines don’t know how to use hydrogen as an aviation fuel because there are no standards and regulations.”
Hydrogen behaves differently than kerosene and will need different fuel assurance and handling processes at airports and airlines. Starting with a small-scale operation using gaseous hydrogen, about which there is much more knowledge than for liquid hydrogen (LH2), is a first step. “This may be a complete revolution in aviation technology, but you still need a stepped approach,” Kavanagh says.
“It starts to help with the hydrogen infrastructure. Because it’s subregional, you don’t have to solve the problem in Glasgow and in London at the same time,” she says. “It is very isolated and small, and that’s good to start with because once you get it over the line, then you’re dealing with the social acceptance part, people touching and flying these hydrogen aircraft. The sooner you start doing that, the better, because it gives investors, the government, the public, the industry confidence this is not a pipe dream.”
ZeroAvia has orders in hand for fuel-cell powertrains to retrofit aircraft from the 19-seat De Havilland Canada Twin Otter to the 70-80-seat ATR 72 and Bombardier CRJ. The U.S./UK startup has thrown its hat into the ring to power Airbus’ 100-seat ZEROe if it goes ahead and is looking beyond to the single-aisle market.
“For introducing hydrogen-electric propulsion into commercial aviation, starting from a smaller scale is much more viable,” Rudolf Coertze, ZeroAvia head of R&D, said at the Farnborough International Airshow in July. The startup’s initial 600-kW ZA600 powertrain for 9-19-seaters uses low-temperature proton exchange membrane (PEM) fuel cells. System-level power density is 1.5 kW/kg, and 60-70 kg (132-154 lb.) of gaseous hydrogen at 350 bar (5,000 psi) gives a range of 300 nm, the company says.
To support a large regional turboprop with 500-nm range requires a 2-3-megawatt powertrain with a system-level specific power above 2 kW/kg and 600 kg of hydrogen, which must now be stored in liquid form. A regional jet could require up to 10 megawatts of power with a power density of up to 3 kW/kg and 1,000 kg of LH2 storage. To address this market, ZeroAvia has launched development of the ZA2000, which is based on its high-temperature PEM fuel cell technology.
Looking ahead to the narrowbody market, ZeroAvia sees the main challenges as fuel cell power density and LH2 storage. A hydrogen-electric Airbus A320 would need just under 15 megawatts per engine and just over 4 metric tons of LH2 for at least 2,400-nm range. This equates to a system-level specific power in excess of 4.5 kW/kg, requiring a fivefold increase in stack-level power density to beyond 10 kW/kg.
“We have shown we can reach a minimum viable power density of 4 kW/kg at the system level. We need even higher than that for the narrowbody market,” Coertze said. “Studies have shown that fuel-cell stack power densities in the region of 10 kW/kg are viable. This is something we are working on, but it takes time, effort and resources.”
As an interim step, ZeroAvia is looking at a single-fuel hybrid engine approach that combines a hydrogen-combustion gas turbine with an electric drive and fuel cells. “This is an interesting configuration that truly gives us potential benefits,” Coertze said, adding that the gas turbine would be used for takeoff and the fuel cells for cruise, reducing fuel burn and improving economics.
“There are a lot of synergies that can be used between the balance of plant that the fuel cells require and the air handling components of a typical gas turbine,” he said. “We see this as a potential steppingstone to help us get toward the overall power densities and power levels required to get into the narrowbody market—and get there sooner.”
A hybrid would need a specific power of 4-5 kW/kg compared with 10 kW/kg for full narrowbody power generation from fuel cells alone. This is closer to the powertrain performance required for a large regional turboprop, which ZeroAvia plans to have on the market in 2027. The startup says a hybrid propulsion system could be ready by the early 2030s.
How hydrogen propulsion technology scales to larger aircraft is a key element of the UK government-backed H2Gear project led by Tier 1 supplier GKN Aerospace. The five-year, £54 million ($71 million) project aims to ground-test a 1-megawatt cryogenic hydrogen-electric powertrain demonstrator in 2026.
H2Gear is supported by the £37 million HyFive project to develop scalable LH2 fuel system technologies, culminating in 2027 with a ground demonstration. And in July, the UK government launched the £44 million H2FlyGHT project to scale the H2Gear powertrain up to 2 megawatts and prepare an integrated propulsion system for flight testing by the end of the decade.
A supplier of aerostructures and engine components, GKN set up a hydrogen systems unit three years ago. Rather than address retrofits, the company decided it could learn more about whether the technologies could meet the targets if it had open clean-sheet platforms onto which to integrate the systems. To this end, while it has no ambition to be an aircraft OEM, GKN created a small internal aircraft design team.
H2Gear is developing a hydrogen-electric propulsion system that uses cryogenic LH2 as fuel and to chill a gaseous helium loop that cools the electrical distribution network, power electronics and superconducting motors to reduce resistance, increase efficiency and save weight. The objective of H2Gear is to not only develop and demonstrate the system, GKN says but identify and resolve aspects that make it scalable and safe.
One of the questions addressed by H2Gear was whether the system should be centralized and located in the rear fuselage or decentralized and distributed in discrete nacelles on the wing. Engineers chose the centralized option, as it allows the system to be housed aft of the cabin pressure bulkhead in a safe zone that is vented continuously to prevent hazardous hydrogen accumulation. “It’s not ‘if’ it’s ‘when’ you have hydrogen leakage,” Norman Wood, GKN technical fellow and aircraft architect, told the American Institute of Aeronautics and Astronautics (AIAA) Aviation conference in Las Vegas in July.
Distribution of hydrogen in any form should be considered with great care and supported by risk and safety analyses, Wood said. The centralized approach does not distribute hydrogen or generate power beyond the unpressurized and fire-protected safe zone. Only power conduits and helium lines cross the aft bulkhead. In the decentralized approach, hydrogen is distributed through the aircraft and power generated at the nacelles. “We have to apply the same safety rules to every nacelle,” he said.
“Centralized is the only way forward to meet the targets,” Wood noted. “Anything that involves distributing hydrogen around the aircraft is incurring risk.” For safety, the fuselage behind the rear pressure bulkhead is separated into three zones with fire boundaries: LH2 storage and conditioning, fuel cell power generation and air management.
“With this approach, the hydrogen is contained within two adjacent zones, which offers the lowest risk of leakage and the highest potential for hazard containment including venting,” GKN says. The team has focused on identifying and mitigating risks such as compressor rotor failure or partial fuselage separation. “Detailed layouts of each zone are progressing with segregation of power, helium and hydrogen lines a key requirement,” the company says.
In GKN’s design, air-delivery compressors are located in the extreme aft fuselage close to the fuel-cell power generation system, forward of which is the thermal management system, then the LH2 tanks mounted behind the rear pressure bulkhead. “Consideration is also being given to access for line maintenance, particularly when the system might be in an operational, cryogenic state,” GKN says.
Stored in dual-walled vacuum tanks at 20K (-253C/-423F), the LH2 is first fed through a heat exchanger to cool gaseous helium to 25K. The helium is delivered first to the motors to cool them to cryogenic temperature. The helium return is then used to cool the DC power conduits from the inverters to the power distribution centers before being returned to the hydrogen heat exchanger.
The intermediate-temperature PEM fuel cells operate at 120C with 50% efficiency, generating electric power and waste heat. Some of the waste heat, plus heat from the helium cooling loop, is used to gasify the LH2 and heat it above 0C for delivery to the fuel cells along with air from the compressors. The remainder of the waste heat is removed by a water glycol cooling system.
An important part of the powertrain is a series-hybrid energy storage system, a combination of batteries to handle power transients and fast-reacting supercapacitors to condition the power supply by removing noise generated by the fuel cell stacks all operating at slightly different voltages. This allows the fuel cells to be operated at near-constant power levels from brake release to top-of-descent and extends their life.
The batteries cover rapid accelerations and decelerations that cannot be handled by regulating hydrogen supply to the fuel cells, Wood said, and are needed to meet European Union Aviation Safety Agency CS-25 certification requirements to be able to go from idle to takeoff power within 8 sec. in the event of a missed approach. The batteries also can provide several minutes of controlled flight with modest thrust if the fuel-cell power system fails.
This combination of hybridization and cryogenic systems provides considerable advantages, he said. Supercooling the power conduits, inverters and motors increases powertrain efficiency downstream of the fuel cells at least 10% over a noncryogenic system. This cancels out the added mass of the cryogenic system, then hybridization reduces overall stack gross power, fuel flow and tank size. Together they reduce block fuel burn up to 20%, the 96-seater using less than 900 kg of LH2 for a 1,000-nm mission.
In the 48- and 96-passenger concepts, the lower fuel burn reduces takeoff weight 3%. The biggest contributors to weight savings are the power conduits, which are smaller, lighter and easier to route through the aircraft. The power cable is housed within a helium conduit that keeps the cable below 75K. The helium conduit is contained within an outer vacuum pipe to minimize heat addition to the helium.
Cryogenic cooling allows high power to be distributed at an aviation-standard ±270 volts via conduits that weigh 40% less than conventional cables carrying the same power at 1.4 kilovolts. The GKN concept aircraft have high wings so helium lines and power conduits can be routed more easily through the upper fuselage and into the wing and any cryogenic helium leaks can be vented clear of the fuselage.
The H2Gear powertrain uses what GKN calls hyperconducting materials, normally conducting metals with high chemical purity that have much lower specific resistivity at cryogenic temperatures. This reduces the DC losses in the power distribution cables considerably.
The power conduits use high-purity aluminum and are 3-4 times lighter per unit length than equivalent conventional ambient-air-cooled copper cables, even including the helium and vacuum containment, Michael Hales, H2Gear chief engineer at GKN, told the AIAA conference.
The same principle is used in the hyperconducting cryogenic motor, which is a partially integrated electric machine and inverter with an ambient temperature iron stator and cryocooled stator coils. The first machine is in testing at H2Gear partner the University of Manchester.
The H2Gear team initially targeted the subregional sector and developed a concept for a CS-23-certified 19-seater. “It’s a difficult place to start, as there is no volume for tanks,” Wood said, adding that external tanks are heavy, draggy and susceptible to damage. GKN moved up to a CS-25-class 48-seater and then a 96-seater and, as aircraft size increased, installation of the propulsion system became easier, Wood said. Recently, the team developed a concept for a 160-seater to show scalability of the centralized system.
The 48-seater has a range of up to 900 nm cruising at 25,000 ft. and Mach 0.45 powered by two propellers and Mach 0.55 with two ducted fans. The propeller version offers an earlier service entry, assuming technology readiness level (TRL) 6 in 2030 versus TRL 6 in 2035 for the ducted-fan version.
The 96-seater has a range of 1,600 nm at 30,000 ft. and Mach 0.6. Twin- and quad-ducted-fan versions were developed assuming TRL 6 in 2035. Both the 48- and 96-seat concepts have standard 3+3 single-aisle seating, a trade-off between higher fuselage drag and greater rear fuselage volume for the 8-megawatt centralized power system and LH2 tanks.
While it is a less mature concept, the four-propulsor 160-seater appears capable of ranges up to 2,200 nm, cruising at 35,000 ft. and Mach 0.7, Wood said. To provide volume for the 16-megawatt power system and tanks, the fuselage cross-section is based on that of the Airbus A330, with 2-4-2 twin-aisle seating.
Integrating the LH2 tanks into the rear fuselage moves the cabin forward and results in greater center of gravity (cg) movement between high and low load factors, and GKN is exploring several schemes to provide safe operation across the cg range.
Early concepts with rear-mounted ducted fans exacerbated the cg range issue, so the team moved to wing-mounted propulsors. “Now that we are confident we can manage the cg range, and given our capability to model scalable ducted fans, we are revisiting the rear fan configurations,” GKN says.
On the 160-seater, folding of the high-aspect-ratio wing is required to fit within the 36-m (118-ft.) span limit at airport gates. While GKN is monitoring development of the NASA/Boeing X-66 transonic truss-braced wing demonstrator, “since we are not constrained by storing fuel in the wing or a requirement for a high transonic cruise speed, there is more freedom to explore alternative concepts,” GKN says.
Around 60% of aviation total CO2 emissions are generated by the 95% of flights that are under 4,000 km (2,200 nm) in range. Having taken on the task of developing and demonstrating a hydrogen-electric propulsion system that would be scalable, the H2Gear team set itself the task of addressing as much of this potential market as possible. Three years in, “we are comfortable with the challenge,” Wood said.